Journal of Soil and Water Conservation, Wntr 2001 v56 i1 p38
Influence of Thermal Gradients on the Rates of Heating and Cooling of Streams. (Statistical Data Included) L.L. Larson; P.A. Larson.
Full Text: COPYRIGHT 2001 Soil & Water Conservation Society
ABSTRACT: Field studies were undertaken to study the influence of thermal gradients on rates of stream heating and cooling. Results from the study on four watersheds suggest that the rates of stream heating and cooling are strongly influenced by exposure to the surrounding thermal environment. Air temperature, when used as an index of the thermal environment, provided a mechanism for estimating the rate of stream heating or cooling in different thermal environments. Rates were tested for significant difference and summarized to provide a framework for anticipating rates of heating and cooling given thermal environment conditions. Monitoring water temperature for watershed assessments determines how much thermalpollution is in a stream. Collecting temperature data between two established monitoring sites, and using air or soil temperatures as an indicator of the watershed's thermal response to climatic factors can help describe why, as well as where, the pollution is occurring. This study evaluates a means of quantifying and evaluating rates of water heating through the application of scientific principles.
Keywords: Stream temperature, thermal gradients
Watershed health and watershed planning is an important issue in the agriculture communities of California, Oregon, Washington, Idaho, and Montana. A primary focus in the planning issue is to regulate agriculture stewardship and project development through state Salmon restoration plans mandated by the Endangered Species listings for species of the Salmonid genus.
Thousands of miles of stream have been put on the Clean Water Act 303(d) list as water quality limited. High water temperature in Salmonid bearing streams is a widespread problem and is assumed to be greater than what the dwindling stocks of spring Chinook, summer Steelhead, and other cold water fish can tolerate. The warmest waters are found in the lower reaches where private landowners conduct agricultural activities.
Most Soil and Water Conservation Districts are involved in facilitating watershed assessments and water quality measurements to determine the impact agriculture is having on water pollution. Landowners in agricultural communities throughout the area are conducting voluntary water quality monitoring on streams crossing their land in compliance with mandatory stream temperature standards and to implement strategies that will keep their water cool. The options considered most often are regulating stream flows and increasing stream shading.
Throughout the Pacific Northwest, data sets have been collected by land managers and watershed councils as part of watershed monitoring programs to determine daily maximum stream temperatures. Many of these programs have relied on published studies to define their program objectives, which include general recommendations for increasing woody vegetation in riparian zones to increase bank stability, stream debris, and provide shade for stream temperature control (Beschta 1991; Beschta and Weatherred 1984; Brown 1969; Brown and Krygier 1970; Oregon Department of Environmental Quality 1996).
Understanding stream temperature is important because it can increase a stream's oxygen carrying capacity and reduce nutrient availability (Lowrance, Leonard, and Sheridan 1985). Some studies have attempted to predict stream temperatures from data collected in areas where vegetation removal may have contributed to stream heating (Beschta and Weatherred 1984; Brown 1969; Brown and Krygier 1970; Theurer, Voos, and Miller 1984). Many of these studies rely on modeling efforts that attempt to describe how stream water temperatures are influenced by solar radiation striking the surface of the stream. Temperature increases due to vegetation removal may adversely affect a stream's biota and generally reduce the stream's fishability (Karr and Schlosser 1978; Lowrance, Leonard, and Sheridan 1985). While these studies have focused on the various amounts of solar radiation impacting stream temperatures, they have not considered analysis of the thermal patterns found in other components of the watersheds, nor the expecte d natural amount of stream heating.
Watershed attributes such as air mass characteristics, elevation gradient, adiabatic rate, channel width and depth, water velocity, surrounding landscape, and inter-flow inputs all influence water temperature and can be of equal or greater importance to stream temperature than vegetation shade (Larson and Larson 1996). A stream flowing through a warm environment will tend to yield a water temperature that reflects the local thermal conditions, and the influence of shade is a part of, nor independent of, that environment.
Soil and Water Conservation Districts and private landowners, who include temperature measurements in their watershed assessments, have been able to determine what the maximum, minimum, and average stream temperatures are each day based on data collected at a specific site. These summaries indicate what the temperatures are as water flows across the thermometers hour by hour. The data reported by thermisters and thermometers are rarely used in conjunction with data collected at a second site beyond comparisons of the maximums for a day. To expand data usage and to determine where thermalpollution occurs, the analysis should be expanded to include a minimum of two sites and a measurement of the velocity of the water between the sites (Larson and Larson 1997). In situations where a monitoring project is designed to account for the variations due to site locations over many reaches of the watershed, velocity of the stream would not be needed.
The velocity of a stream can be used to determine what the water temperature change is throughout the day based on how many miles the water flows during the testing period. For example, in early spring the velocity of a river could cause water to move fast enough through a system to travel 12 mi in four hours. In late summer the velocity would be less and the water would pass through that same 12 mi over a 12 hr period.
Data collected on thermisters record the energy accumulations in stream water, air, or soil for the site where they are placed. Water flowing across the thermister one hour may change temperature but to know the temperature change during the second hour, a second site must be located downstream where the water has moved.
Data analysis of measurements recorded at a single site can be compared to multiple sites if a strategy is used to account for the variations in the data due to site location. Temperature measurements recorded at various sites from mountain headwaters to valley bottoms can be used together to describe the thermal patterns and account for the influence of the changing landscape of a watershed. In this situation the sites might be located many hours apart and each site would represent a portion of the stream profile. Using multiple sites in this design would enable land managers to compare thermal cycles that are influenced by factors of location. An obvious and natural method is to use topographic elevation to select sites.
This study describes stream temperatures at 10 sites in four watersheds in Oregon. The study focuses on expanding the quality of the information gained from stream temperature data by describing the changes in temperature during diurnal heating and cooling periods related to site locations. The focus is on the change in temperature and the rate of stream heating and cooling associated with thermal gradients and topographic elevations. This methodology can be used by land managers to anticipate the amount of temperature change associated with orographic influences.
Materials and Methods
Field studies were conducted in June 1995 through August 1997 in four watersheds (10 sites total) in Eastern Oregon. Table 1 summarizes the site elevations and years of data collection. Stream sites were randomly selected within watersheds by topographic elevation from 2,123 m to 914 m above sea level to reflect the vertical stratification of (1.9-3.0[degrees] C 304 [m.sup.-1]) of the thermal environment (Riehl 1972; Shaw 1982; Trewartha 1968). Two sites were selected on each stream that were separated by at least 304 m.
The headwaters for streams (2,123 m) are located in Lodgepole pine forest areas and are derived from springs and a series of marshy seeps. Midelevation sites are located in mixed conifer forests of grand fir (Abies grandis), Douglas fir (Pseudotsuga menziesii) and Pondersoa pine (Pinus ponderosa). Low elevations are located in agriculture croplands.
Stowaway temperature loggers (Onset Computer Corp., Pocasset, MA) were used to measure water and air temperature. Stowaway temperature loggers have a manufacturer specified accuracy of [+ or -] 0.2[degrees] C and were used to monitor temperatures at one hour intervals. A total of 10 sites were selected for the experiment.
Data were collected at four sites in two different watersheds during 1995. Data collection was expanded in 1996 to include two additional sites in a third watershed, and a fourth watershed in 1997. Data were summarized in July and August during 1995, 1996, and 1997.
Daily mean thermal gradients were calculated by averaging the differences in temperature observed between air and water at each thermister data point over a 12 hr heating or 12 hr cooling period (described in The Laws of Thermodynamics).
Air temperature was used as an indication of the thermal environment of the watershed. Accumulated degree hours (Miller and Donahue 1990; Richardson and Leonard 1980) were calculated using the equation
ACDH = [[[sigma].sup.p].sub.i = 1] [T.sub.i]
where: ACDH = the accumulated degree hours for the period of the day being studied.
P = Period of Accumulation = the number of hours per day studied.
[T.sub.i] = the temperature of the thermal body for hour i of the study period.
Using degree hour accumulations in both air and water temperature data sets, linear equations were calculated for each day during the heating (5 A.M. to 5 P.M. Standard time) and cooling (5 P.M. to 5 A.M. Standard time) periods described above (1,160 equations). Coefficients of determination from this analysis were above 95% with slope estimates significant at p [less than or equal to] 0.05. Linear equations provided a time average of the daily thermal response recorded by the data loggers, and accounted for the thermal lag within the system between air and water heating.
The data collected at each site was summarized using two types of numeric data for each thermal period; the rate of thermal accumulation (the slope of the linear equation) and the mean thermal gradient of a daily heating or cooling cycle. Each day's heating and cooling rate was coupled with the daily mean thermal gradient and tested by analysis of variance. The data were then grouped by elevation to determine the influence of orographic location.
The validity of this grouping was evaluated through discriminant analysis (Stat Graphics 7.0). Ten observations were selected randomly from each of the appropriate groups and discriminant separation was tested.
Scientific Principles
Average surface/air temperatures typically lag four to eight weeks behind maximum solar radiation, reflecting the annual pattern of energy accumulation in the earth's thermal environment and subsequent redistribution by climatic patterns (Trewartha 1968). This is reflected in the Northern Hemisphere by the occurrence of mean monthly temperatures, which are highest in July and August, rather than at the time of peak solar radiation (the summer solstice).
The heating of a natural body of water is governed by two primary radiation sources: the sun, and the ambient radiation emitted by the atmosphere and the earth (Larson and Larson 1996). Whether there is net radiative cooling or warming depends on the balance between emission and absorption. Infrared radiation is emitted continuously, and emission rates are usually greater during the day than at night (Bohren 1987). The surface of the earth receives more energy from the atmosphere than it does from the sun even though the sun is hotter. The sun doesn't cover as much of the sky as does the atmosphere and the radiation coming from the direction of the sun isn't as much as the reradiated energy from the atmosphere above us. The atmosphere plays an important role in the heating of the planet (Bohren 1987).
Heating and cooling cycles are dependent on the amount of time that there is direct solar radiation, and the time that there is none. Both periods have thermal gradients between the various components in a watershed and their surrounding environment. There are two fundamental theories that support the association of thermal accumulations with thermal gradients: the Laws of Thermodynamics and the heating and cooling due to atmospheric vertical stratification as reflected in orographic elevation.
Thermodynamics. The Second Law of Thermodynamics (Halliday and Resnick 1988) states that energy exchange occurs from areas of high concentration toward areas of lower concentration and that the rate of exchange (how fast energy accumulates or dissipates) will depend on the magnitude of the temperature gradient.
Edinger et al. (1968) described the energy exchange between water and meteorological conditions by predicting the magnitude of the thermal gradient that exists between water and an equilibrium temperature (net energy exchange = 0) at the air-water interface. The equilibrium temperature served as a descriptor of the thermal environment, a reference point to estimate the thermal gradient, and as a basis for estimating the rate of energy exchange.
In this study the difference between air temperature and water temperature at a given hour was used as a descriptor of the thermal gradient. The greater the magnitude of this gradient the faster water will heat, and the smaller the magnitude the slower the rate of heating.
Figure 1 shows air and water heating and cooling at two elevations on the same stream during a 15 hr period. All of the watershed is being heated at the same time with the coolest environments in the upper reaches of the watershed. Development of a thermal gradient begins at dawn and changes hourly, maintaining the potential for heating. The thermal gradient at the upper elevation site shows the air temperature at dawn greater than the water temperature and a positive thermal gradient exists. The gradient between air and water temperatures at dawn at the lower elevation site is negative. Even though there is solar radiation at the lower site, air temperatures are less than water temperatures, and until a positive thermal gradient is established the water temperatures remain unchanged. Air temperatures at both sites increase steadily from dawn until midafternoon and water temperatures lag behind due to the high specific heat of water and the requirement of a positive thermal gradient for thermal exchange betwe en the two bodies.
During the day, the air mass reached maximum temperature at the upper site and lower site over a period of six hours and nine hours, respectively. Water temperatures increased 6[degrees]C at both sites, but the increase at each site differs in the number of hours contained in the temperature increase cycles. The upper site reached maximum water temperatures at 2 P.M., held the maximum until 3 P.M., and then decreased. The lower site reached maximum at 4 P.M., held the maximum until 6 P.M., and slowly decreased over the next several hours (data not shown). Maximum thermal gradients at the lower site occurred at 2 P.M. and became smaller during the next few hours. This slowed the rate of water temperature increase from 1 to 1.5[degrees]C increase per hour to 0.5[degrees]C or less each hour. The cycle at the upper site was steady throughout the day and water increased 1[degrees]C or greater each hour until 3 P.M. Maximum air temperatures produced the greatest thermal gradient, and when air temperatures dropped, the gradient declined and continued to decline as night descended and the entire watershed cooled down.
Recognizing the important role thermal gradients play in the cycle of heating and cooling in a watershed allows us to examine how much potential there is for heating at a given hour and throughout the daily cycle. At the upper elevations, there is a very large gradient compared to the lower elevations throughout the day, but the upper elevational environment cools earlier in the day than the lower site. If it did not, it is likely that the headwater temperatures of mountain streams, located at high elevations, would increase more than at the lower elevations. Figure 1 demonstrates how, as a large thermal gradient decreases, the rate of water temperature increase is slowed.
Water temperature is expected to increase throughout the day because it is governed by the thermal gradients that occur between the air mass and water body at a site. Comparing the rates of heating or cooling when grouped by elevation, describes the natural thermal heating of the environment in a watershed. The data analysis in this study, by applying the principles of thermodynamics, determined how fast the water heated between two sites located at points with a difference in elevation of at least 304 m.
Orographic influence. Vertical heating and cooling due to elevational changes in topography is also called "adiabatic" heating and cooling. Where mountains are present, temperatures decrease with increasing altitude at a rate near 1.9-3.0[degrees] C 304 [m.sup.-1] (Shaw 1982). Adiabatic influences in air masses are generally described using data collected at two sites separated by at least 304 m elevation difference. Larson and Larson (1997) described the pattern of heating and cooling for water moving down an elevational gradient and the scientific principles associated with the heating and cooling.
Journal of Soil and Water Conservation, Wntr 2001 V56 I1 P38
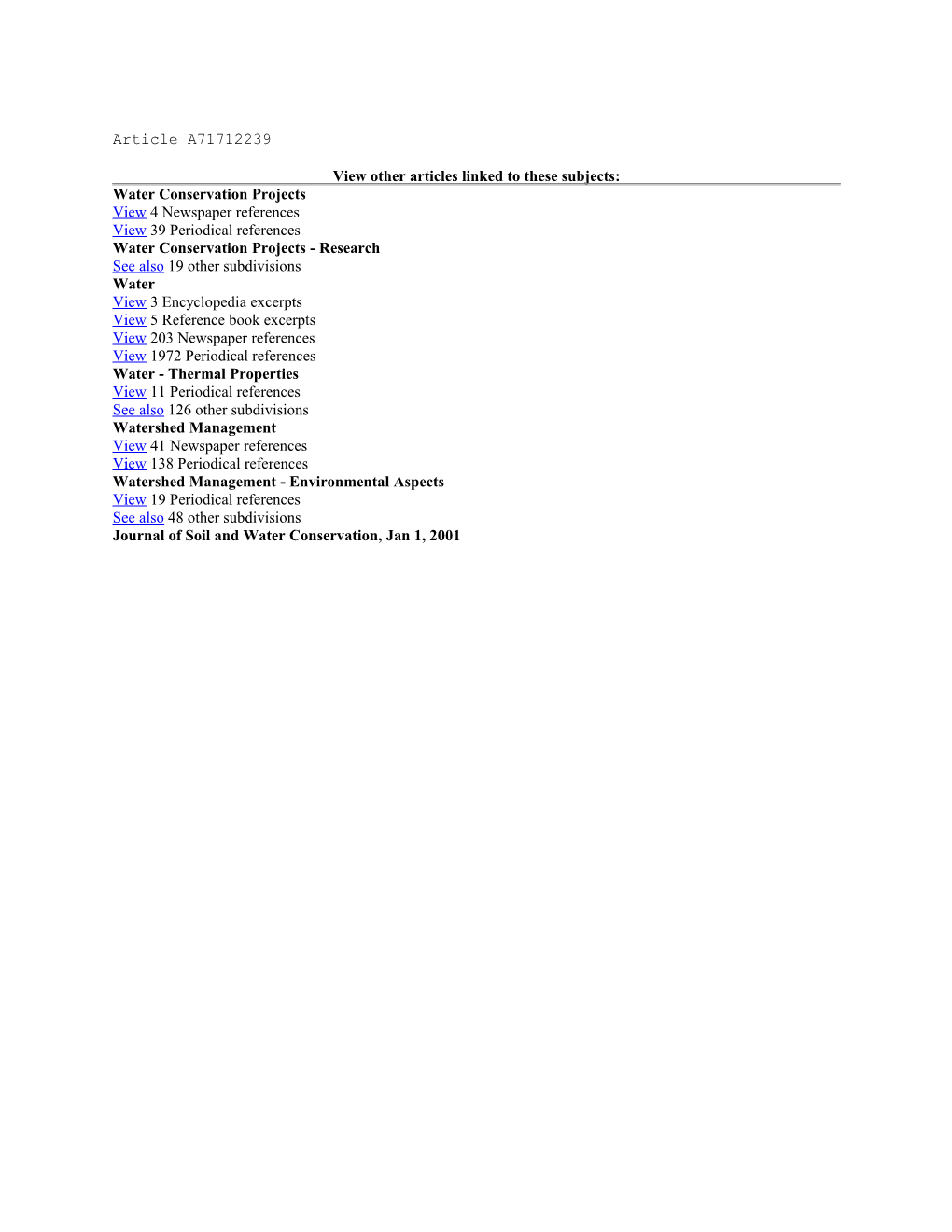